核融合科学コース
プラズマ実験
大型の核融合実験装置を用いた燃焼条件に近い高温プラズマや、小型装置による基礎プラズマの実験的研究指導を行います。
大型ヘリカル装置(LHD:Large Helical Device)
![]() |
核融合科学研究所の中心的なプラズマ閉じ込め実験装置です。2本1組の超伝導ヘリカルコイル(紫色の部分)と、6本3組の超伝導ポロイダルコイル(黄色の部分)により最大約3テスラの閉じ込め磁場を生成し、ドーナツ状のプラズマ(薄紫色の部分)を閉じ込めています。プラズマの大半径は3.5~4.1 m、小半径は約0.6 mです。ヘリカル型のプラズマ閉じ込め実験装置としては、世界最大の大きさです。プラズマは中性粒子ビーム入射(NBI:Neutral Beam Injection)加熱、電子サイクロトロン共鳴加熱(ECRH:Electron Cyclotron Resonance Heating)、イオンサイクロトロン周波数帯(ICRF:Ion Cyclotron Range of Frequencies)加熱などによって最大約1億度にまで加熱されます。燃料の水素は、固定ペレット入射法とガスパフ法の2種類によって供給されます。また多くの計測装置も装備されてます。 |
プラズマ物理・制御
磁場閉じ込め方式での核融合炉実現のためには、高温(~1億度)・高密度(~1020 m-3)・長いエネルギー閉じ込め時間(~1秒)のプラズマを生成し、維持することが必要です。ここでは、このような炉心プラズマを制御する基本要素(磁場配位、壁条件、排気、燃料注入)に関する研究を行います。すなわち、プラズマの平衡・安定性、輸送過程、熱・粒子バランス等の物理機構の解明と、それに基づいた核融合炉心プラズマ生成、温度・密度・不純物の制御、磁場構造やプラズマ粒子の注入・排出、壁材料との相互作用等を含めた炉心プラズマの総合的制御システムとしての観点から教育研究を行います。
(a)高密度・高温プラズマの生成
ヘリカル系閉じ込めで実現される無電流プラズマは電流駆動を必要とせず、高密度に起因する破壊的不安定性(ディスラプション)がないため、トカマクと比べて高密度運転に優れています。高密度運転はプラズマ対向壁損耗を軽減するなど、核融合炉の工学的要求の解決を容易とするとともに、ヘリカル系に特有な新古典拡散の制約を軽減することができます。したがって、ヘリカル型核融合炉の高密度運転シナリオの確立に向けた、無電流高密度プラズマの高性能化は重要な課題です。
LHDの最近の実験により、高効率の排気と中心燃料供給によって、内部拡散障壁が形成され、1021 m-3を超える超高密度プラズマが得られました。この成果は高密度核融合炉心運転に新しいシナリオを提供するものです。一方、この内部拡散障壁形成の物理機構、その炉条件への適用性、超高密度プラズマの運転限界などについての課題が解決すべき事項として具体化してきています。
また、高いイオン温度を実現することは、核融合を実現する上で必要不可欠です。LHDではこれまでに9400万度の中心イオン温度を得ることが出来ました。さらに、このような高いイオン温度が実現している時には、プラズマの回転速度が空間的に大きく変化していることが観測されており、イオンのエネルギー閉じ込めとの関連が調べられています。また、中心イオン温度が高くなると中心での不純物炭素の量が減るという不純物の輸送に関する興味ある現象も観測されました。
(b)平衡配位・磁気流体力学的不安定性の研究
核融合プラズマは、磁力線でつくられたカゴ(平衡配位)によって閉じ込められます。平衡配位は、プラズマの閉じ込め性能および磁気流体力学(MHD:Magneto Hydro Dynamics)特性とお互いに関連しています。高いプラズマ閉じ込め性能や良好なMHD特性を持つプラズマを得るためには、最適な平衡配位を維持することが要求されます。さらに、より高いベータ値(β値:プラズマ圧力と磁気圧力との比)を得るための最適な平衡配位の探求が進められています。LHDでは磁気軸位置やプラズマ半径等、平衡配位の最適化を計って年々最大β値を更新してきており、最近では、5%の目標値を達成するに至りました。高温・高密度のプラズマでは、自らが磁場を横切って逃げる不安定性を起こすことが知られています。β値が3%を超えるあたりからMHD不安定性が観測されていますが、閉じ込めへの影響はほとんどなく、β値は上昇を続け、破壊的な不安定性なしに閉じ込め時間の50倍程度の長時間にわたり安定な高β値を保持することができました。理論研究では、平衡配位の詳細な計算がなされており、実験結果との比較を通じて詳細に研究されています。
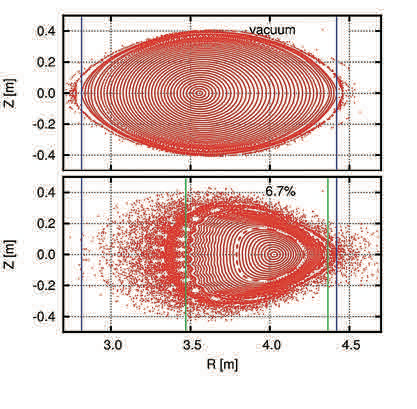
(c)対向壁材料・プラズマと壁との相互作用・ダイバータの研究
核融合研究の歴史上、閉じ込め改善は全てプラズマ対向壁の状態改善やダイバータ磁場配位の採用など、周辺プラズマ制御の進歩によって得られています。また核融合炉実現上の最難関課題の一つは炉壁だと言われています。核融合炉では、炉心プラズマから周辺に膨大な熱・粒子の流出が予想されますので、高性能な炉心プラズマを維持しつつ熱・粒子流を制御してプラズマ対向壁・ダイバータ板の損耗・損傷を防ぎ、かつヘリウム灰除去や燃料供給などの粒子制御をしなければなりません。したがって周辺プラズマやプラズマ・壁相互作用は大変重要な研究課題です。
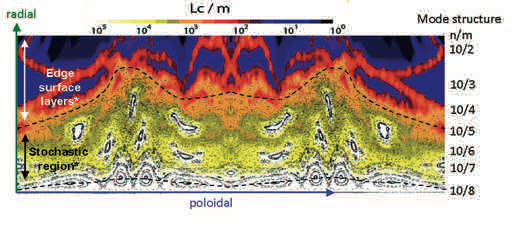
プラズマ対向壁への熱・粒子負荷の軽減のためには周辺プラズマ中における熱・粒子輸送過程の理解が必須です。また、プラズマ対向壁の損耗・損傷、不純物の発生と輸送、燃料粒子のリサイクリング等はプラズマ制御および対向壁の健全性確保のための重要課題です。プラズマ・壁相互作用に関する研究としては、放電洗浄やコーティングによる壁コンディショニング法の評価および第一壁・ダイバータ板の損耗、再堆積過程の理解とその制御等が挙げられます。これらは、核融合炉を想定した場合の長時間放電の際に特に問題となる課題であり、磁場閉じ込め核融合装置共通の課題です。その解決を通じて、国際熱核融合実験炉ITERを含む次期核融合装置の設計や運転計画立案に寄与しています。
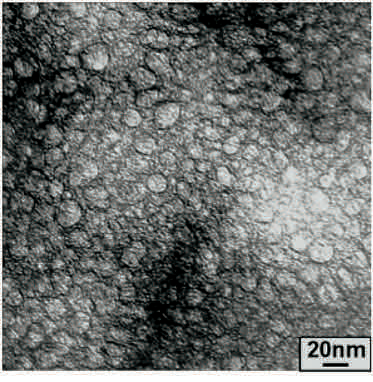
透過型電子顕微鏡で撮影したプラズマ対抗壁(ステンレス鋼)内部に形成された高密度ガスバブル
(d)プラズマの制御に関する研究
超高密度コアプラズマの実現と長時間維持可能な閉じ込め改善運転のためには、磁場配位・密度・加熱などの制御が必要となります。
磁場配位制御の一環として、MHD不安定性の回避をしつつプラズマの高ベータ化を目指すことを目的として、放電時間中に磁場配位を実時間で変化させる実験が開始されました。将来の高ベータ化へ向けた新しい知見が得られる可能性を持った研究が進められています。また、密度制御の手法である燃料供給法には、大きく分けて次の2種類があります。1つは常温の水素ガスを吹き込む方法(ガスパフ)、もう一つは低温の固体水素の塊を高速で打ち込む方法(ペレット入射)です。LHDでは、微小流量から大流量(0.01~240 Pa m3/sec)までの様々なガスパフをプレプログラム制御あるいは密度フィードバック制御で使用できるほか、高速水素ペレット入射装置や連続水素ペレット入射装置など多彩な燃料供給装置が装備されています。
これらの燃料供給法を効率よく運用することで、粒子輸送の研究に資するほか、超高密度プラズマや高ベータプラズマの生成などに貢献しています。
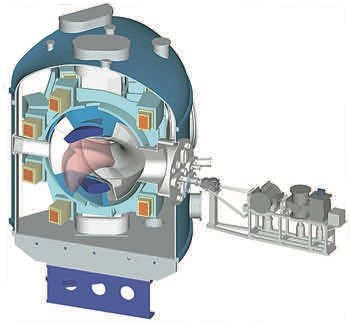
プラズマ加熱
高温高密度プラズマの生成・加熱について、プラズマ物理および装置工学の両面から研究します。プラズマ物理として、プラズマ生成・加熱ならびに電流駆動の機構、大電力加熱・電流駆動の閉じ込め性能への影響について、また同時に装置工学として、高効率プラズマ加熱のための中性粒子ビーム生成方法、電磁波の発生・伝送・入射方法等の最適化の研究ならびに、これらの加熱装置の開発に向けた、大型イオン源、大電力粒子ビーム輸送技術等の開発、大電力高周波源、伝送系要素部品、高性能アンテナ等の設計・開発の研究を行います。
代表的なプラズマ加熱の方法には、高速の中性水素原子ビームをプラズマに入射させて、そのエネルギーでプラズマ中のイオン及び電子を加熱する中性粒子ビーム入射(NBI)、高周波の電磁波をプラズマに入射させて、プラズマ中のイオンまたは電子を選択的に共鳴加熱させる高周波加熱があります。後者には、電子のサイクロトロン運動に共鳴させて加熱する電子サイクロトロン共鳴加熱(ECRH)、イオンのサイクロトロン運動を利用して加熱するイオンサイクロトロン周波数帯ICRF)加熱などがあります。これらの加熱法により、高温プラズマの生成・加熱をはじめとして、プラズマ中に電流を駆動することも行います。
(a)中性粒子ビーム入射(NBI)加熱
NBIでは、高速の中性粒子をプラズマに入射させて加熱します。高速の粒子ビームは、イオンを電気的に高エネルギーまで加速すれば生成することができますが、プラズマは磁場により閉じ込められているため、外部から荷電粒子を入射させることができません。そこでイオンビームを電気的に中性化してから、プラズマに入射させます。入射した中性ビームは、プラズマとの衝突により再びイオン化されて、磁場に捕捉され、プラズマを加熱します。イオンビームの中性化には水素ガスを用いますが、中性化効率により、核子当たり100 keV以上の高エネルギー領域では負イオンを、それ以下の低エネルギー領域では正イオンを用います。
NBIは大型ヘリカル装置(LHD)における主加熱装置です。負イオンNBIが3台、正イオンNBIが2台の5台が取り付けられており、入射加熱電力の総計は27 MWです。NBI加熱によりこれまでに、1.6 MJのプラズマ蓄積エネルギー、5%を超えるベータ値、9400万度のイオン温度、1.2×1021 m-3のプラズマ密度等を達成しています。
- NBI加熱プラズマのプラズマ閉じ込め特性と輸送の解明
- NBI加熱により達成された高イオン温度プラズマの特性についての研究
- NBI高エネルギーイオンの閉じ込めと損失機構の解明
- NBI高エネルギーイオンが励起するMHDモードとその相互作用の研究
- 入射高エネルギーイオンのプラズマ中での減衰過程の解明
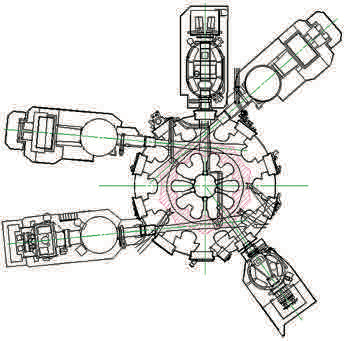
- 負イオン源プラズマの分光的測定
- 負イオンの生成機構とプラズマからの引き出し機構の解明
- ITER-NBIシステムへ向けた高周波負イオン源の開発
- 高エネルギーイオンの中性化に関する研究
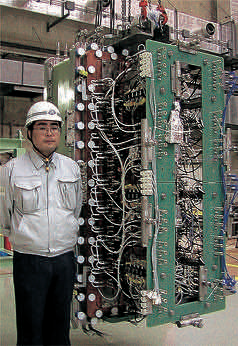
(b)電子サイクロトロン共鳴加熱(ECRH)
ECRHはミリ波を用いてプラズマ中の電子を加熱する手法です。ジャイロトロン装置と呼ばれる発振器によって生成されたミリ波は100 m程度の伝送路を通り、最終的にLHD内部に設置されたアンテナからプラズマに入射されます。
LHDでは1 MWを超えるミリ波出力が可能なジャイロトロン装置が5台あり、最大5.6 MWのミリ波電力をプラズマに入射することができます。ミリ波はアンテナの角度を制御することによって、プラズマの様々な位置に入射することができるため、加熱だけではなくプラズマ中の電場や閉じ込め磁場の制御にも使用されます。
これまでに、ミリ波をプラズマ中心領域に精度良く入射することにより、電子の熱閉じ込め改善を実現し、2億度を超える電子温度を達成しています。また、ECRHによって1時間を超えるプラズマの定常保持も実現しています。
- ECRHプラズマの閉じ込め特性
- 電子内部輸送障壁の形成機構
- ミリ波電流駆動がプラズマ閉じ込めに及ぼす影響
- 電子バーンシュタイン波を用いたプラズマ加熱
- ECRHで生成される高エネルギー電子の振る舞い
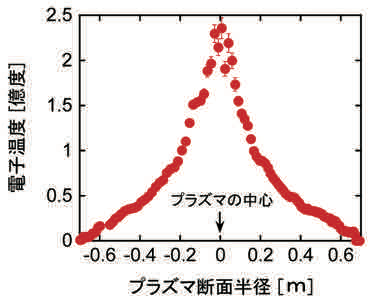
- ジャイロトロンの大電力化
- ミリ波伝送システムの高効率化
- 入射アンテナシステムの高速化・高精度化
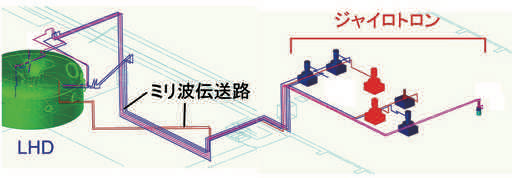
(c)イオンサイクロトロン周波数帯(ICRF)加熱
ICRF加熱では、イオンサイクロトロン周波数またはその近傍の周波数である数十MHzの周波数帯の電磁波を用いて、主にイオンの加熱を行います。サイクロトロン運動をしているイオンを単純に共鳴的に加熱することは、高密度プラズマでは難しいため、プラズマ中に伝搬する波動を介して、二種イオン加熱、高調波加熱等によるイオン加熱を行っています。また、静電波へのモード変換によるイオン・バーンシュタイン波加熱等、電磁波とプラズマの相互作用に関わる研究も行っています。
LHDでは、二種イオン加熱、水素イオンの2次高調波加熱、モード変換による電子加熱、イオン・バーンシュタイン波加熱等の多様な加熱実験に応じた周波数が使われています。高周波源には四極真空管を用いた発振増幅器が4系統あり、同軸管によりLHD本体室へ伝送されます。高周波は整合回路を経て、LHD真空容器内に設けられたアンテナからプラズマへ入射されます。アンテナは3対6本あり、これまでに4.5 MWの加熱電力を達成しています。多様なICRF加熱実験を行っており、加熱モードと加速された高エネルギーイオンの閉じ込めの研究を行っています。また、1時間程度の定常プラズマ加熱と保持を達成しており、その時の加熱入力エネルギーは3.36 GJという世界最高値となっています。
- LHDにおける多様なICRF加熱プラズマの閉じ込め特性の解明
- ICRF加熱により加速された高エネルギーイオンの閉じ込めの研究
- モード変換による電子加熱の研究
- 定常加熱と定常プラズマ保持に関する研究
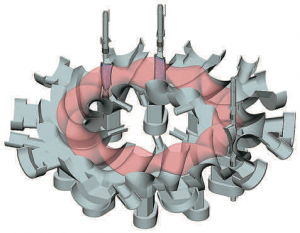
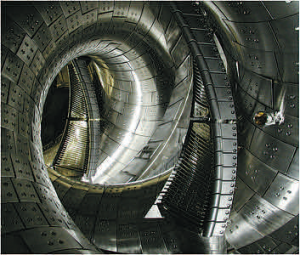
プラズマ計測
プラズマ物理を深く探究し核融合炉を実現するためにはプラズマ特性を正しく計測することが重要です。プラズマ温度、密度、閉じ込め時間の基本パラメータをはじめ、多くの物理量が対象になります。現在、LHDには多くの種類の計測装置が取り付けられています。LHD実験では、これらの計測装置を同時に動かすことによってプラズマの様々な情報を得ることができます。このような多くの情報を総合的に解析することによって核融合プラズマの性能を向上させる道筋が見えてくるのです。
高温プラズマの計測においては静電プローブのようにプラズマに直接計測器を挿入する計測は限られた範囲でしか行うことができません。 したがって高温プラズマ計測は、プラズマから放射される電磁波、粒子などを計測する受動計測、プラズマにレーザー、マイクロ波、粒子ビームなどを入射してプラズマからのリアクションを計測する能動計測の2種類に分類することができます。
- 磁気揺動計測
- 電子サイクロトロン輻射計測
- 高エネルギー粒子計測
- 可視分光計測
- X線計測
- 重イオンビームプローブ計測
- マイクロ波反射計測
- トムソン散乱計測
- 協同トムソン散乱計測
- 遠赤外レーザー干渉計
- ダイバーター干渉計
- 荷電交換分光計測
- トレーサペレット入射による計測
プラズマ計測技術は、常に新しいアイディアや最新のテクノロジーにより発展し続けています。
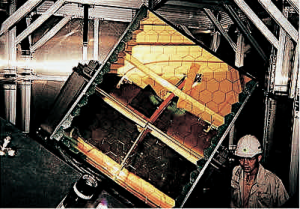
プラズマイオン温度計測
核融合プラズマでは温度計測のため、プラズマから出てくる光の情報を用います。プラズマからは様々なエネルギー(色)を持った光が出てきます。この中から温度の情報を持つ光だけを取り出すため、「分光器」と呼ばれる機器を用いて光をエネルギースペクトルに分解します。取り出した光のスペクトル広がりから、プラズマの温度が計算されます。プラズマ温度計測は、核融合の実現に私達がどれだけ近づいているかを知るために、欠かせない計測器です。
イメージング計測技術
プラズマの外で得られる情報だけでプラズマ内部の様子を知ることは、とても難しいことです。しかし近年のプラズマ計測技術は、この問題を解決し現在ではプラズマ断面の物理量の1次元的な分布を同時に計測できるようになりました。さらに分布が時間によって、どのように変化するのか、ということも測定可能になりました。
最新の計測技術発展の成果は、このような1次元計測から、さらに進んで核融合プラズマの2次元分布を、写真撮影したように同時に計測するイメージング計測が可能になったのはCCDなどの高性能な2次元検出器と大量のデータを瞬時に解析することを可能にした情報処理技術が両輪となって発展したことによります。イメージング計測は、我々に核融合プラスマの複雑な様相を垣間見せてくれます。
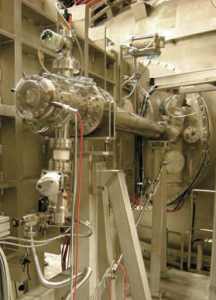
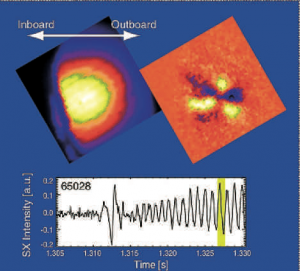
プラズマ計測は現在も発展し続けている研究分野です。まさに未開の荒野を開拓していくチャレンジングな世界であり、新しい発見に出会える興奮が、そこにはあります。また近年、プラズマ技術がナノテクノロジーなどの産業分野に盛んに応用されるようになってきましたが、そのような最前線の舞台でも核融合科学研究所で開発されたプラズマ計測技術が重要な役割をはたすことでしょう。
プラズマ基礎
核融合科学研究所では、LHD実験、数値シミュレーション、炉工学を3本の柱として研究が進められていますが、他にもいくつかの支援装置があり、プラズマ科学分野全般において有用となると考えられる基礎的な研究を全国の大学との共同研究として行っています。
小型装置によるプラズマ基礎実験では、大型装置ではなかなか実行できない、プラズマを直接目で見て、手で触れて実験を進めることができるという魅力があります。将来、核融合プラズマ研究の道へ進むことを希望している方にとっても、学生時代にこのような規模の小回りの効く装置で実験を行うことは非常に有意義な経験になるでしょう。もちろん経験だけでなく、これらの小型装置を用いてプラズマ基礎分野での新しい発見も可能ですし、それを目指して全国の大学の共同研究者との研究が進められています。
HYPER-Ⅰ装置におけるプラズマ基礎実験
HYPER-Ⅰ装置は周波数2.45 GHz、最大出力80 kWのマイクロ波源を用いて、直径30cm、長さ2m、電子密度1019m-3の高密度定常プラズマを生成することができます。磁場配位は弱発散型で、プラズマは電子サイクロトロン共鳴過熱によって生成されますが、このときマイクロ波を右回り円偏波で磁力線に沿って強磁場側から入射することで正常波モードのカットオフ密度を大きく上回る高密度プラズマを得ることができます。
こうして生成されたプラズマは荷電粒子の集合ですから電気的・磁気的な相互作用によって様々な集団運動を形成します。また大規模な流れの空間パターン(渦)を自発的に形成することがあります(図2)。
これらの渦構造の形成機構を理解するには、プラズマの温度、密度、電位分布、速度場等、様々な物理量を計測する必要があります。HYPER-Ⅰ装置ではこれまで各種ブローブを用いた粒子計測を主に行なってきました(図3)。最近ではレーザーを用いた分光学的計測に力を入れ始めています。レーザー計測ではプラズマに対する擾乱が小さく、流速の絶対値が得られるという利点があります。また、中性粒子の計測も可能となるため、これまで電子・イオンといった荷電粒子のみのダイナミックスを考えていた現象に対して、中性粒子の流れとの相互作用といった新しい観点からの研究の進展が期待されています。
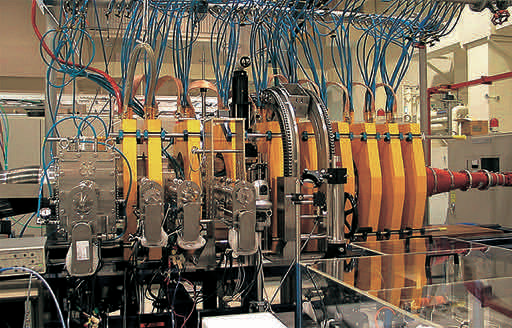
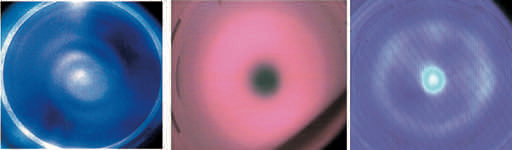
(左)銀河の渦巻きのような”スパイラル渦”
(中)台風の目のような密度ホールを伴った「プラズマホール」
(右)明るく光る渦がEXBドリフトとは逆向きに回転する「反EXB渦」
炉工学
超伝導マグネット開発研究
核融合炉工学の分野では、核融合炉の2つの重要な構成要素、高磁場生成のための超伝導マグネットシステムと、発電、燃料増殖の機能を担うブランケットシステムの基礎、応用、開発研究を行っています。高温、高熱負荷、大電流等の極限状態における先端技術開発を通して、核融合炉以外の幅広い分野への応用を視野に入れた学際的な研究教育を展開しています。
ヘリカル型核融合エネルギー炉のシステム設計に関する研究については、国内および国際共同研究を通じて、将来のヘリカル型核融合エネルギー実証炉の設計研究を強力に推進しています。具体的には、核融合炉システム設計、ヘリカル磁場配位の最適設計、超伝道マグネットシステムの工学設計、電磁力支持構造物の工学設計、ブランケットの工学設計、など多岐に渡り、いずれも、学術的に最先端の研究内容となっています。
超伝導・低温工学研究
磁場核融合装置に必須である大型・高性能の超伝導マグネットシステムのために必要となる基礎研究や応用研究を行っています。LHDは、世界最大の超伝導マグネット・低温システムを有しており、プラズマ実験のために連続した安定な磁場を供給しているだけでなく、装置工学実験を遂行することによって、その性能をさらに向上させてきています。また、研究所では、将来のヘリカル型核融合エネルギー実証炉の設計活動を進め、その巨大な超伝導マグネット・低温システムの設計に取り組んでいます。より高性能な超伝導コイルと高効率の低温技術をめざし、次世代の超伝導材料や線材、大電流容量導体を研究開発するとともに、コイルの巻線技術、冷却技術、電源技術、等に関して、幅広い基礎研究や応用研究を行っています。これらの技術には、核融合炉以外の応用も視野に入れて研究が行われています。
LHDを用いた装置工学研究
LHDを実際に用いた装置工学実験を行い、LHDの超伝導マグネット・低温システムの特性について詳細に研究することで、核融合炉用大型システムに必要な設計条件を最適化する研究を行っています。また、超伝導マグネット研究棟や総合工学実験棟における実験装置を利用して、超伝導導体やコイルの実験、要素開発研究などを行って、LHDの超伝道マグネット・低温システムのさらなる性能向上にも努めています。
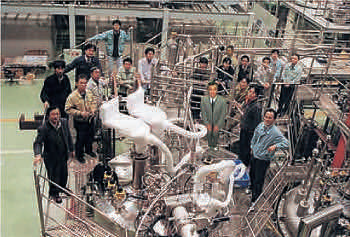
核融合炉用超伝導マグネットに用いる大型導体の開発研究、導体特性や電磁現象等に関する研究
核融合炉の大規模超伝導マグネットシステムには100 kA級の大電流先進超伝導導体が要求されます。そこで、金属系低温超伝導材料であるNb3Alや、酸化物系高温超伝導材料のY系やBi系などの線材を最適に組み合わせた大電流導体の設計研究を行っています。また、導体設計をもとに実際に短尺導体サンプルを試作し、通電試験を行うことでその特性を詳細に調べます。線材や導体には、超伝導材料と周囲に用いられる常伝導金属の双方においてさまざまな電磁現象が現れます。これらを詳細に研究することは優れた導体開発を行なっていくうえで欠かせません。併せて、大型超伝導導体では、冷却安定性と常電播現象に関する研究が安全な通電を行なうために重要な課題となっています。
(左)強制冷却ケーブル・イン・コンジット方式NbTi導体
(中)間接冷却方式Nb3Sn導体
(右)間接冷却方式酸化物系高温超伝導導体
先進的な超伝導材料と長尺線材開発のための基礎研究
核融合炉の超伝導コイルに用いられる線材の超伝導材料に関して、更なる特性向上をめざした基礎研究が重要な課題となっています。また、これらの材料を用いて長尺線材を作るための応用研究が不可欠です。
核融合炉マグネットにおける巨大な電磁力支持構造物や大型導体の機械・材料特性に関する研究、大型超伝導コイルの製作方法に関する開発・設計研究
核融合装置の大型超伝導コイルでは、巻線導体やコイル容器に巨大な電磁が印加されます。電磁力によって材料にかかる応力を正確に評価し、いかに安全に支持するかは極めて重要な研究課題です。特に、核融合炉では装置の軽量化も必須の課題であり、最適設計が求められます。合わせて、大型の超伝導コイルをいかにして巻線・製作するかという工学設計も重要な研究課題となっています。
中性子環境下における超伝導材料の特性研究
核融合反応によってプラズマ中で生じた高速中性子は、超伝導コイルの内側に置かれた厚いブランケットによって、約10万分の1まで強度が弱められます。しかしながら、最低でも約30年と考えられている核融合炉の運転期間中に微量の中性子が超伝導コイルに当たり続けると、その特性が変化してしまうことが懸念されています。そこで、中性子環境下における超伝導コイル用材料の特性を研究するとともに、中性子環境下における使用に適した新しい先進超伝導材料やコイルシステムの開発を進めています。
大型ヘリウム冷凍機やサブクールシステム、 先進的な小型冷凍機の技術開発に関する研究
核融合炉の巨大なマグネットを冷却するためには、現在までに開発されている大規模なヘリウム液化冷凍機をさらに性能向上させることが要求され、既存技術の深化が重要な課題となっています。このために、計算機シミュレータを構築することなども重要な研究テーマとなっています。併せて、低温における物性に関する研究や、超流動ヘリウムやサブクールヘリウムの物性・熱輸送に関する研究なども興味深いテーマとして進めています。
一方、超伝導技術を民生応用としてさらに発展させるためには、効率の良い冷却技術の開発が求められています。このために、先進的なパルスチューブ冷凍機の開発も進めています。
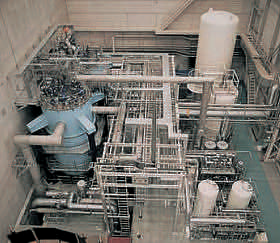
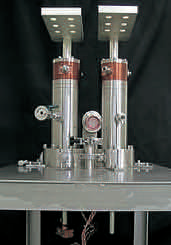
レーザー核融合のためのクライオターゲットの開発に関する研究、および、低温物性に関する研究
レーザーを用いた慣性核融合の爆縮用ターゲットとして、燃料となる水素を極低温で氷らせたクライオターゲットの開発研究を行なっています。均質なターゲットをいかに効率良く生成するか、低温物性研究を駆使した最先端の応用研究となっています。
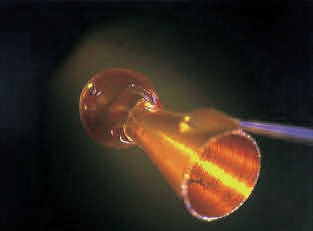
大型超伝導コイルシステムのための電源制御システムに関する研究
核融合装置の大規模超伝導コイルシステムには外部から電源によって大電流が供給されます。特にヘリカル型装置における超伝導コイル群は、電磁的に密に結合しているため、各コイルの電流値を精密に、かつ、高速に制御することは重要です。このために、最新の制御理論を駆使した電源制御システムの構築が研究課題となっています。
大型超伝導コイル・低温システムの産業応用に関する研究
超伝導コイルシステムは核融合だけでなく、幅広く電力機器への応用を視野に入れて開発研究を行なっています。当グループでは、水素併給型超伝導送電ケーブルや超伝導エネルギー貯蔵装置の開発研究などについても、積極的に取り組んでいます。また、先進的な冷凍機技術と関連して、環境に優しい熱音響エンジンの開発研究も行なっています。
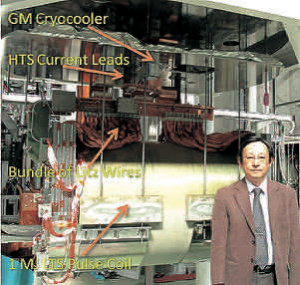
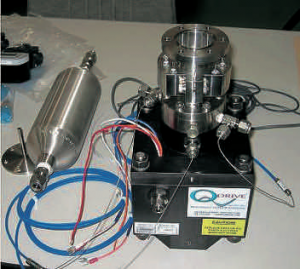
先進ブランケット開発研究
核融合炉では、炉心の外側をブランケットと呼ばれる構造物で覆い、このブランケットにより核融合反応で生じた中性子を熱エネルギーに変換し、発電を行ないます。溶融塩や液体金属を冷却材兼燃料増殖材に用いる超寿命型の先進ブランケットに関する学術研究をしています。
核融合炉ブランケットシステムのニュートロニクス評価
ブランケットには、炉心プラズマで発生した高速中性子を受け止めて、「熱への変換」、「トリチウム燃料増殖」、「放射線遮蔽」を効率よく行うことが要求されます。そのために最適な材料の組み合わせや配置、ヘリカル型発電炉に適したブランケット形状等の研究を中性子輸送計算により進めます。また、日本原子力研究開発機構との共同研究により、高速中性子照射による設計検証実験を進めます。
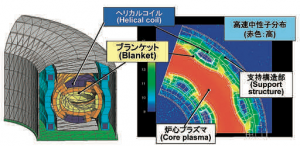
(右)炉心の中性子分布計算結果
炉材料の開発研究
ブランケットに用いる構造材には、中性子照射によって生じる誘導放射能の減衰が速い材料(低放射化材料)を用いる必要があり、代表的な候補材であるバナジウム合金の開発に取り組んでいます。低放射化バナジウム合金共通材料(NIFS-HEAT、NIFS-HEAT2)を製作し、大学と協力してその特性評価とコンポーネント開発に向けた試作研究、特性の更なる改良に向けた基礎研究などを進めています。
(中)高温における材料の強度を評価可能なクリープ試験機
(右)実験中の様子
液体ブランケットの材料共存性に関する研究
ブランケットの自己冷却型とリチウム増殖材として期待されている溶融塩フリーベ(LiF-BeF2)や液体金属リチウム(Li)中の材料腐食現象を明らかにする為に、高温における共存性試験や強制流動型腐食試験用装置の要素技術研究・設計研究を進めています。
(右上)溶融塩中へ低放射化フェライト鋼JLF-1(9Cr-2W) を浸漬した結果
(下)実験装置の前で
ブランケットにおける機能性材料に関する研究
液体ブランケットシステムにおいて、高磁場で流動させた場合に磁気流体力学(MHD)的効果により、流動を妨げる圧力損失が生じます。また化学的なプロセスにより、材料腐食が生じたりします。これを抑制するために、セラミックス絶縁被覆材料の開発を行っています。また、ブランケットの中を流れる溶融塩や液体金属中の燃料ガスの濃度を、固体電解質セラミックスセンサを用いて測定することに挑戦しています。
(右)プロトン導電性固体電解質を応用した水素センサー
放射線・安全工学
磁場核融合実験施設における安全、とりわけ放射線安全に関する研究を行ないます。磁場核融合装置が社会に受容されるには、放射線安全に関する研究の展開が不可欠です。将来の核融合炉ではトリチウムを燃料とし、核融合反応に起因して中性子線が発生します。発生した中性子線によって装置や周辺機器が放射化されます。このような状況で、水素同位体の回収・濃縮と環境負荷低減、作業環境における線量計測と放射線防護、電磁場環境の安全評価、施設周辺の環境影響評価、そしてシステム安全設計が研究課題として挙げられます。これらの課題は、核融合科学研究所で行われている重水素プラズマ実験における放射線安全とも関連しています。
放射化作業環境における放射線防護に関する研究
核融合反応の結果生じた中性子は、装置や周辺機器を放射化します。放射化によって作業環境に放射線源が分布することになります。作業計画を立てるにあたって、線源の時間的空間的な変化を把握する必要があります。
線源分布の把握は、計算による予測と測定によって行います。放射化の状況を放射線輸送計算によって導き出し、それを線源として線量分布を算出します。測定では、遠隔にある放射線源分布の可視化装置の開発も進めます。計算と測定の両面の結果を用いて、放射線防護計画を策定します。
水素同位体の回収・分離に関する研究
将来の核融合炉では、水素同位体が燃料として使用されます。この水素同位体を回収し、同位体を分離、再利用する「燃料サイクルシステム」を構築することは、核融合炉の実現に向けて、重要な課題です。
水素同位体の回収方法として、酸化吸着法、膜分離法を研究しています。一例として、電気化学反応により水素を選択的に透過する高温型固体電解質膜を用いて、不純物ガス中から水素同位体を回収する手法を開発しています。比較的大型の試料を用いて、水素同位体の回収特性の把握、ならびに電極などを工夫して性能の改善を目指しています。実験は、名古屋大学 同位体分離実験室に設置された装置を用いて行われます。
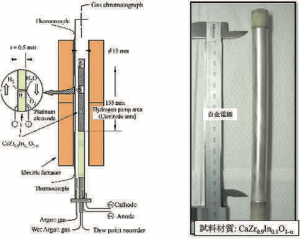
(右)プロトン伝導性酸化物試料
水素同位体ガス処理用ハニカム型触媒・吸着剤の特性研究
将来の核融合施設における安全問題として、燃料として用いるトリチウムの漏洩対策があります。先進的な漏洩トリチウム除去システムの開発に向け、圧力損失低減と大流量処理に適するハニカム型触媒・吸湿剤の基本性能を基礎的、体系的に調べる必要があります。
ハニカム材として使われているセラミックス系と金属系について、基材の製作方式や表面処理によって変わる特性変化をミクロ、マクロ的な視点から調べます。
トリチウム計測システムの開発研究
核融合炉の燃料であるトリチウムの計量管理や施設内の安全管理、環境影響評価の観点から、トリチウム計測システムの開発は重要な研究課題です。トリチウム計測では、トリチウムが大量に存在する燃料サイクルシステムから、極々微量の環境レベルまで、20桁におよぶ範囲を計測対象としなければなりません。広範囲の濃度を計測するためには、トリチウムの物理化学特性を理解し、計測に応用することが必要です。
この研究では、低濃度のトリチウム計測を目的に、システム開発に取り組んでいます。方法の一つとして、電離作用を利用した検出システム開発を行っています。ここでは、トリチウムによるβ線の信号と、他の放射線や電気ノイズによる信号を、電気回路を工夫して波形弁別することを目指しています。トリチウムを用いた実験は、名古屋大学 同位体分離実験室を利用して実施します。
その他の主な研究テーマ
- 核融合炉の放射線安全システム設計に関する研究
- 磁場核融合実験施設における電気安全・システム安全に関する研究
- 磁場核融合実験施設の電磁場環境と安全に関する研究
- 先進的な放射線監視システムの開発研究
- 放射線遮へい解析に関する研究
- トリチウムの安全取扱い技術に関する研究開発
- 環境モニタリング設計に関する研究
- 環境中トリチウム挙動に関する基礎研究
- トリチウムを含む有機物試料の迅速処理に関する開発研究
- 核融合システム内の水素同位体挙動に関する研究
- マイクロ波によるトリチウム回収用吸湿剤の再生処理研究
- 大気圧プラズマジェットを利用した水素化合物の燃焼研究
核融合から21世紀の産業技術へ
核融合科学研究所では、これまでに培った大電力マイクロ波技術を基礎として、マイクロ波の電場と磁場が物質を構成する原子分子の内部にある電子に直接働きかけることを見いだしました。この原理を使うと、水やセラミックスなど非導電性の物質だけでなく、金属であっても粉末、つまり不連続体ならば、マイクロ波で効率よく加熱できることを明らかにしました。外部からの熱に依らない物質の創成と新しい加工技術の誕生です。
これまで人類が1万年にわたって使ってきた「炎」という古典的手段に対し、物質の電子構造に直接働きかけるというマイクロ波は、各国の研究者や産業界の注目を集めています。
マイクロ波によるセラミックスの焼成
長年に渡る地元との共同研究によって、マイクロ波を用いた陶磁器焼成の工業化に世界で初めて成功しました。この研究は、現在、地元の中部地区だけでなく、全国の企業・研究機関・大学との共同研究に発展しています。マイクロ波焼結の迅速性により、窯業におけるエネルギー消費を数分の一に低減でき、地球環境の保全に役立つと期待されています。また、国立天文台との共同研究によって、大型望遠鏡の高度な光学技術と結合させて、新しいゼロ膨張セラミックスを開発することに成功しました。これは、半導体製造などにおける革新技術として産業界の注目を集めています。
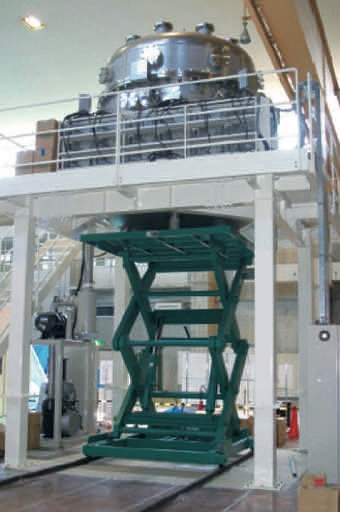
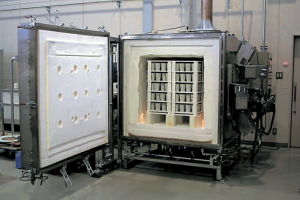
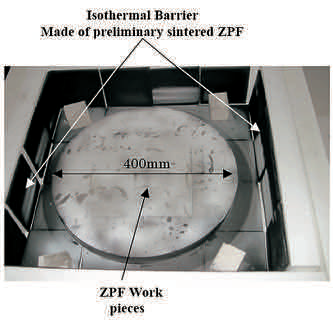
マイクロ波による鉄の精錬
東京工業大学と核融合科学研究所の共同研究によって、鉄鉱石と石炭の混合粉末にマイクロ波を照射して銑鉄を作ることに成功しました。昇温と還元のエネルギーをマイクロ波の電磁場エネルギーで供給しますので、供給炭素量を1/2にすることができ、全産業比として炭素ガス排出量を4%削減できると期待できます。また、この方法によって得られた銑鉄は、不純物濃度が通常の高炉から得られる銑鉄の1/10という高品質なものでした。
理論・シミュレーション
核融合プラズマのような複合複雑系で発生する現象を総合的に理解していくためには、実験装置を用いた研究とともに、理論・シミュレーション研究の果たす役割が大きく、とりわけスーパーコンピュータを駆使した大規模シミュレーション研究は、他の学問分野に先駆けて発展してきました。本コースでは、シミュレーション研究に必要な様々な手法や科学的可視化研究を教育するとともに、プラズマ物理の理論体系化を目指した教育研究を行います。さらに、複合複雑系で発生するすべての物理過程を取り込み、閉じ込めスケールサイズでの実験結果の予測を可能とする総合的シミュレーション体系の構築を図ることにより、シミュレーション研究を新しい科学分野として確立・発展することを目指してゆきます。
磁場閉じ込めプラズマシミュレーション
LHDをはじめとする磁場閉じ込め装置内のプラズマは、様々な要素が絡み合い非常に複雑な挙動を示します。そのため、その挙動をよく理解することは、閉じ込めプラズマの実験予測や提案のために非常に重要となります。シミュレーション研究では、以下に挙げた具体的な研究課題を通じて、磁場閉じ込めプラズマの複雑挙動の解析を行っています。
LHD実験プラズマの磁気流体シミュレーション
LHDにおける崩壊現象や非線形緩和現象を対象として、磁気流体力学(MHD)などの流体モデルを用いたシミュレーション研究を行い、プラズマの巨視的構造変化について解析を進めます。そして、実験結果に対する解釈や従来の安定性の概念に対して、新たな示唆を与えることを目指します。具体的には、シアフローが交換型モードの安定性に与える影響、反磁性効果を含むMHDシミュレーション、共鳴摂動磁場と圧力駆動型モードとの相互作用等について、教育・研究を行います。また、新たな数値シミュレーションコードの開発や、計算結果の可視化手法などについても研究指導を行います。
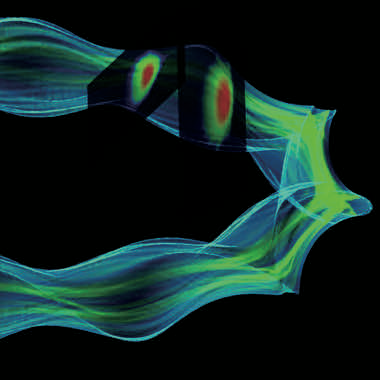
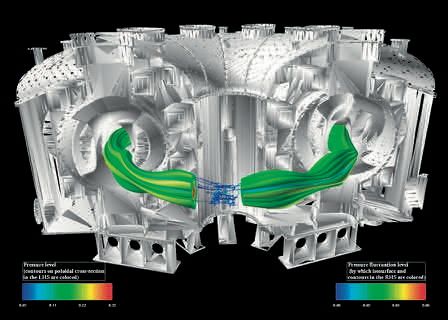
高速粒子と磁気流体モードの相互作用
核融合プラズマにおける磁気流体と高エネルギー粒子の相互作用およびそれを解明するための粒子・流体統合シミュレーションについて研究及び教育を行います。磁気流体・高エネルギー粒子相互作用は高速アルファ粒子が発生する核燃焼プラズマにおいて特に重要な研究課題であると同時に、磁気流体波動やランダウ減衰・成長などのプラズマ物理の主要な課題を含んだ興味深い問題です。
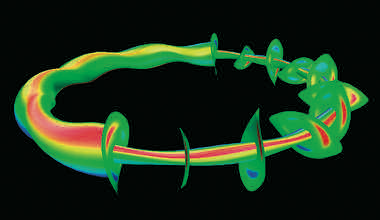
核融合プラズマの乱流輸送現象の解明
磁場閉じ込め核融合プラズマ研究の重要課題である粒子及び熱の乱流による輸送現象の解明を目標に、大規模数値シミュレーションを駆使した研究を進めています。乱流輸送の予測には、ゾーナルフロー等の自己組織化を通じた輸送抑制機構に代表されるプラズマ乱流の非線形性を理解する必要があります。そのために電磁場と相互作用する5次元位相空間における粒子分布関数を追跡するジャイロ運動論シミュレーション(または3次元ジャイロ流体シミュレーション)を行います。さらには核融合プラズマにおける密度及び温度分布を予測するために、大規模乱流シミュレーションで得られた知見を活用し、本質的な支配物理の抽出と乱流輸送モデルの構築に取り組みます。
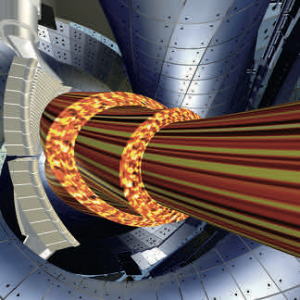
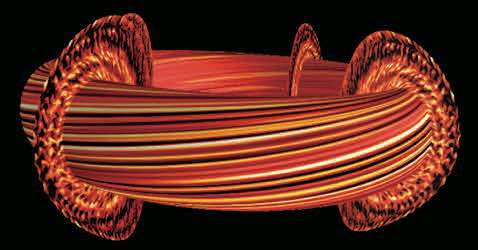
核融合プラズマにおける新古典輸送現象の解明
3次元トーラス磁場に閉じ込められた荷電粒子の複雑な運動とクーロン衝突は新古典輸送現象と呼ばれる粒子・熱流束を生み出します。閉じ込め磁場の形状(ヘリカル、トカマクなど)や温度密度に応じて大きく特性が変化する新古典輸送と、それによってプラズマ内に自発的に形成される両極性電場をドリフト運動論に基づき自己無撞着に解き、プラズマ閉じ込め性能の評価や、両極性電場の乱流輸送への影響、新古典粘性がプラズマ回転に与える影響などの理論・シミュレーション研究を行います。
ヘリカル系の周辺プラズマ輸送
磁場閉じ込め核融合プラズマにおける周辺プラズマ輸送、特に3次元トーラス磁場配位における磁気島を含む磁力線構造が乱れた領域での輸送現象を対象に、数値シミュレーションによる研究を行っています。具体的には、流体モデルやドリフト運動論に基づく熱・粒子・中性粒子・不純物輸送シミュレーションを通して、ダイバータ領域の輸送現象を研究しています。また、数値シミュレーション手法の開発およびその基礎研究について、シミュレーション科学に関する幅広い視野からの教育・研究を行います。
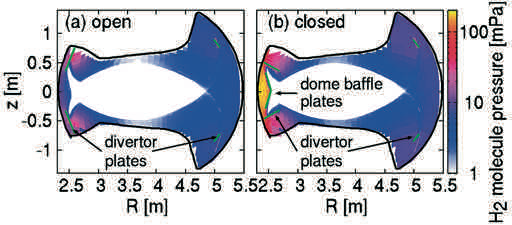
その他の主な研究テーマ
- トカマクプラズマの輸送シミュレーション
- 多相拡張磁気流体モデルによる固体水素の溶発シミュレーション
- 磁気流体平衡の拡張
- 高ベータプラズマシミュレーション
- LHD周辺磁場構造の理論解析
磁場閉じ込めプラズマ理論解析
現在、そして将来において計算機性能が飛躍的に発展したとしても、複雑なプラズマの振る舞いを逐一再現することは非常に困難です。従ってシミュレーションと現実のプラズマとをつなぐ理論モデルの構築は不可欠です。ここでは理論解析に関する研究課題を紹介します。
プラズマ輸送現象に関する理論解析
磁場閉じ込め核融合プラズマを記述する運動論方程式や流体・輸送方程式の定式化やそれらに基づいた輸送現象の理論研究を行います。3次元トーラス磁場配位プラズマにおける電場・流れ場形成や微視的不安定性に関する解析や、Lagrangian/Hamiltonianを用いた現代的定式化による対称性や保存則に関する理論解析、さらには運動論的性質を取り入れた流体方程式系の完結モデルの考案に取り組みます。
磁気流体モデルに基づく閉じ込め理論解析
プラズマの流体モデルを用いて、MHD及び核融合プラズマ閉じ込め理論について研究を進めています。特に、3次元磁場配位を対象として、等方圧力分布を持つ静止MHD平衡の解析及び非等方圧力分布や流れのある平衡への拡張、MHD線形スペクトラム(安定側及び不安定側)の解析、流れや自発電流を中心とした新古典拡散理論との融合、2流体モデルへの拡張及び運動論的効果の導入等について、理論解析や数値計算手法の開発を行います。さらに、LHD実験結果を用いて、理論と実験結果との比較を行い、理論の妥当性についての検討を進めます。
閉じ込め改善モードに関する輸送解析
プラズマ閉じ込めに大きく影響を与える電場構造や流れのような物理現象の解明を目的とします。核融合プラズマ実現に重要な、閉じ込め改善モードについて輸送研究を行います。新古典輸送が決める電場の非線形性により、電場構造には遷移(分岐)現象が見られます。乱流輸送に対する遷移現象の影響について定式化や解析研究に取り組み、閉じ込め改善モードの実現を目指します。
複雑性プラズマシミュレーション
プラズマをはじめとする複雑なシステムにおいては、時として自己組織化現象や間欠性エネルギー解放現象といった共通の物理現象が現れます。このような複雑性に起因する物理の諸課題に取り組むことにより、新たな理論の構築を目指します。そこで得られる知見は、核融合プラズマに限らず、天文や材料など広範な分野への応用が期待できます。
3次元開放系での無衝突磁気リコネクションのダイナミックス
プラズマ中における構造形成のダイナミックス・自己組織化現象に関する大規模シミュレーション研究を進めています。具体的には、1)ミクロ階層とマクロ階層を繋ぐ無衝突磁気リコネクションの多階層シミュレーション、2)電流層におけるプラズマ不安定性と異常抵抗発生機構に関する粒子シミュレーション、3)エネルギー開放系におけるプラズマ構造形成のダイナミックス、4)磁場閉じ込めプラズマ中で発生する構造形成・自己組織化の物理等に関する教育・研究を行います。
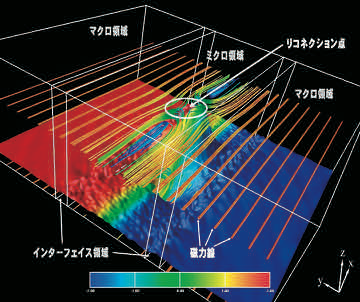
粒子シミュレーションによるプラズマ基礎過程の解明
プラズマ粒子シミュレーションにより境界層プラズマ基礎過程を解明する研究を行っています。特に、壁近傍のプラズマ挙動、中性粒子とプラズマの相互作用等の関与したプラズマ構造形成等についてスーパーコンピュータを駆使して探求しています。
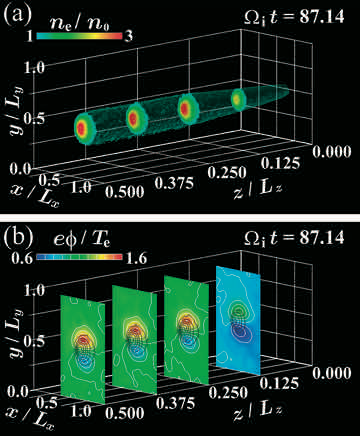
分子動力学シミュレーションによる原子スケールの研究
核融合装置内壁に代表されるプラズマと物質の相互作用が引き起こす様々な現象を数値シミュレーションによって解明しています。分子動力学法、密度汎関数理論、二体衝突近似法、動的モンテカルロ法など種々の計算手法を時空間およびエネルギーのスケールによって使い分けるマルチスケール解析を行っています。最近では、これらのコードを組み合わせたハイブリッド手法を開発することで、タングステンの繊維状ナノ構造など、個々の手法では再現が難しい現象の探求も積極的に進めています。
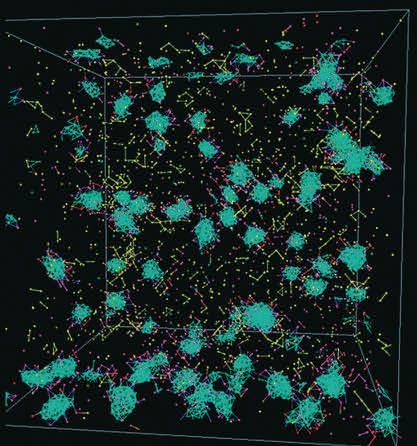
その他の主な研究テーマ
- 水素と炭素材との衝突過程の分子動力学シミュレーション
- 衝撃波による粒子加速
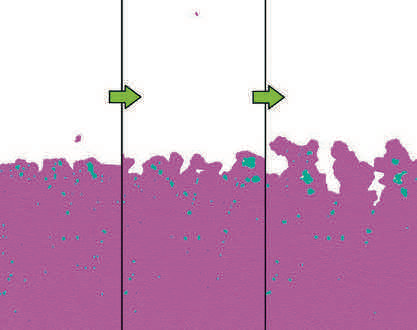
シミュレーション手法開発
シミュレーション研究を遂行するためには、現実の物理世界をいかにして数値的に表現するか、その膨大な数値データをいかに効率よく計算処理して、得られた結果をいかにして人間が理解できる形で表現するかといった手法に関する課題に取り組む必要があります。本コースでも既存の手法の枠組みを超えた挑戦的なシミュレーション手法の開発に力を入れています。
バーチャルリアリティシステム
様々な複雑現象に関する数値シミュレーションで得られる結果は、一般に非常に複雑な時空間構造を持っています。そこで複雑な形状をありのまま3次元空間内で表示可能な、没入型バーチャルリアリティシステムの開発を進めています。4面がスクリーンで囲まれた部屋の中に観測者が専用のセンサー付き眼鏡をかけて入ることにより、高度な没入感が得られ、知的思考の助けとなります。このシステムを利用することにより、数値解のもつ複雑な構造を理解することのほか、実験装置を本物そっくりに再現して装置の最適化への利活用も進めています。
その他の主な研究テーマ
- 先進的なシミュレーションシステムの構築
- 高精度・高効率数値計算スキームの開発
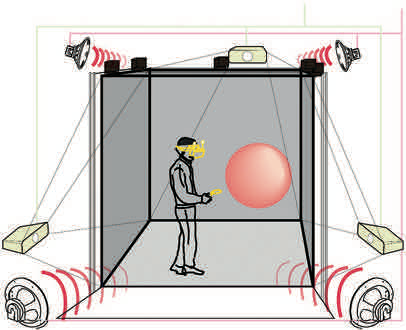
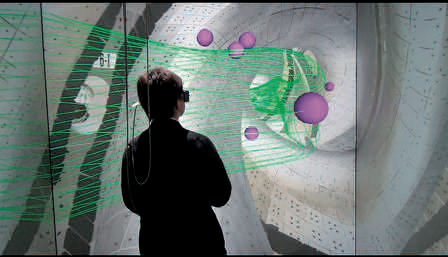
プラズマシミュレータ雷神
核融合プラズマ閉じ込め物理機構解明とその体系化、それを支える基礎研究としての複雑性科学の探求、シミュレーション科学の確立をめざした共同研究を支援するため、核融合科学研究所に設置されたシステムです。前述のバーチャルリアリティシステムをはじめ、シミュレーションの実行からデータ処理まで、高速ネットワークを介したシミュレーションに関する共同研究を一貫して効率よく推進するための総合的なシステムとして「プラズマシミュレータ雷神」と名付けられました。基幹となる大規模並列型計算サーバは令和2年7月に更新されたNEC社製SX-Aurora TSUBASA(4,320ベクトルエンジン(VE))で、総VE主記憶容量202TiB、総VE演算性能10.5PFlopsのスーパーコンピュータです。
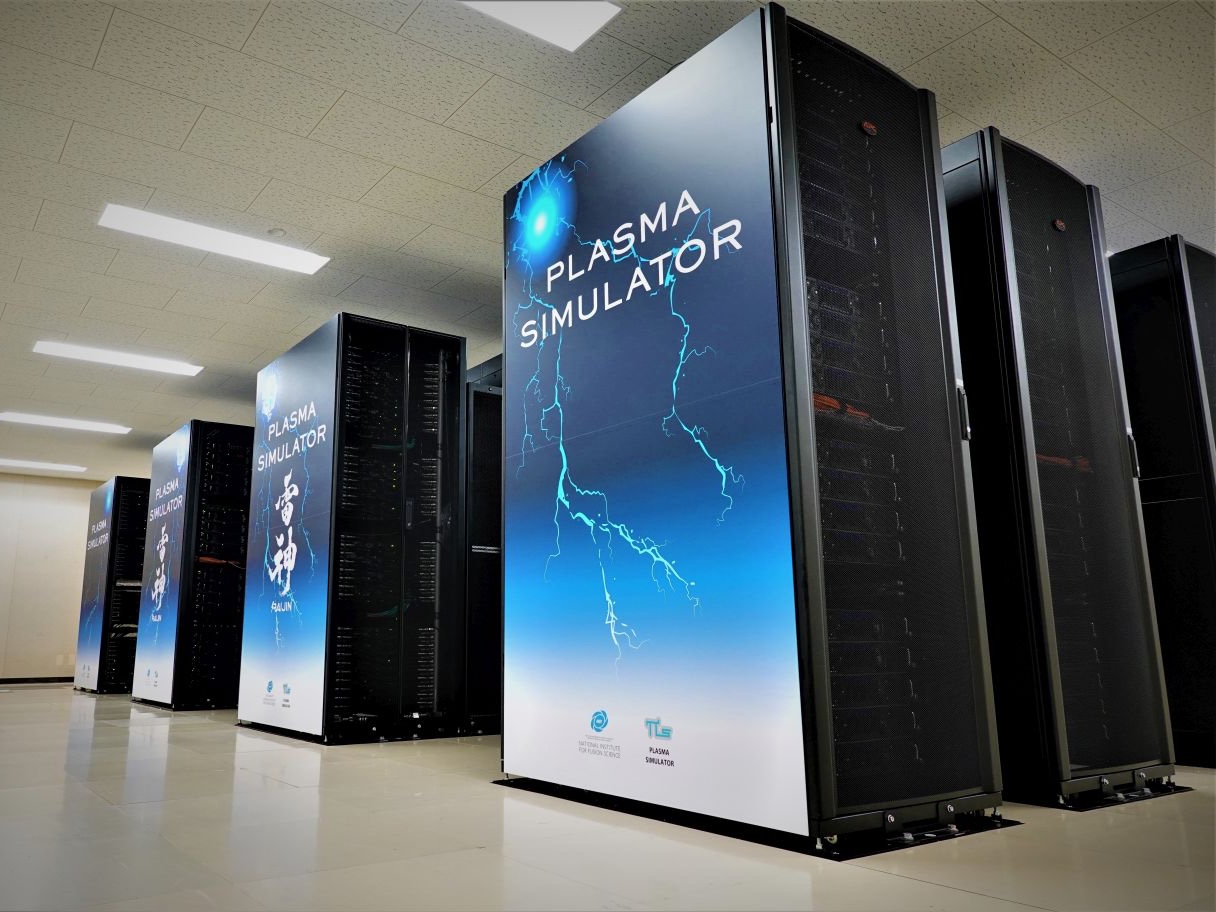
SX-Aurora TSUBASA
4,320VE
202TiB(総VE主記憶容量)
10.5PFlops(総VE演算性能)
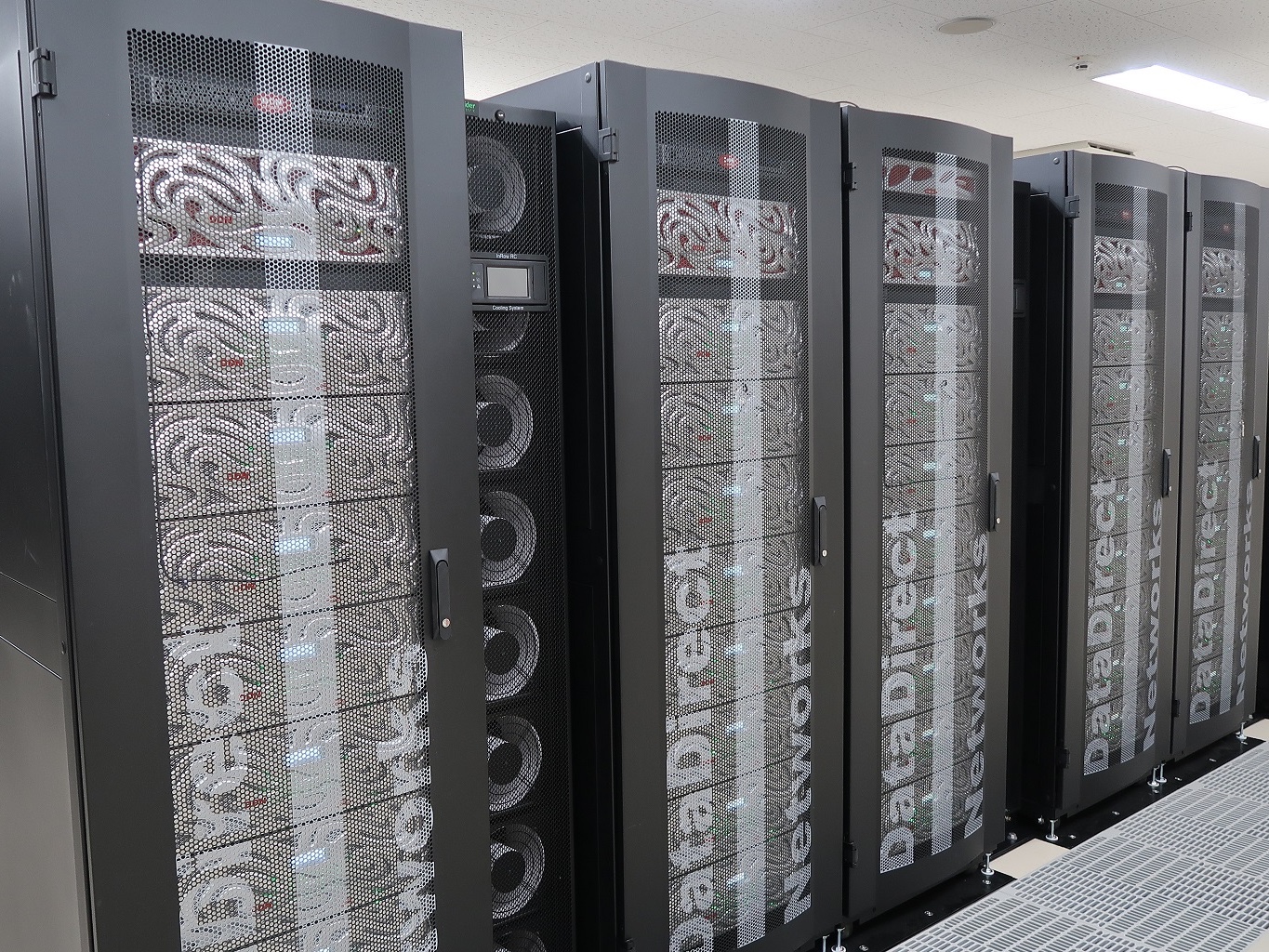
32.1PB